Essential Insights on Power Semiconductors in Electric Vehicles:
- The process of generating the power required by Electric Vehicles (Electric Vehicles) does have environmental implications.
- The integration of Wide Bandgap semiconductors, such as Gallium Nitride and Silicon Carbide, into Electric Vehicles optimizes their electrical efficiency.
By using Wide Bandgap semiconductors, Electric Vehicles can draw less power from the grid, contributing to a more sustainable future.
Introduction
The emergence of the Electric Vehicle (EV) is a significant factor in today’s efforts to reduce CO2 emissions, conserve energy, and protect our environment. The driving force behind EVs is electricity. For an EV to fulfill its role as an environmental champion, it must consume as little grid power as possible. This is achieved by maximizing energy efficiency, a task made possible by wide bandgap semiconductors (WBGs), such as gallium nitride (GaN) and silicon carbide (SiC).
Before we delve into the workings of WBGs, let’s briefly explore two automotive ecosystems.
Gasoline vs Electricity
Indeed, Electric Vehicles do have an edge! Electric motors are inherently more efficient than their gasoline counterparts.
Take a look under the hood of a powerful vehicle from the 1960s – you can see the ground beneath the vehicle! In contrast, a modern gasoline vehicle’s under-hood space is filled with a complex array of pumps, tubes, vents, and other components – a testament to pollution control.
This is absent in Electric Vehicles.
Electric motors don’t produce pollution like gasoline engines – no need for a pollution control system. All hydrocarbon pollution control is managed at the source – the power plant. Instead of millions of gasoline-powered cars, each equipped with a complex, energy-consuming automatic pollution control system, there’s just one such system at the power plant, continuously monitored by human experts and sophisticated automation. The result is a single highly effective system replacing millions of less efficient vehicle-based systems.
The Electric Vehicle’s Energy Storage
The EV’s “fuel tank”, its power storage, is the lithium Ion Battery (LiB), which can store around 150 kilowatt-hours of DC electricity at 800 volts. However, very few components in the car operate at 800 volts DC.
The main motor operates at AC voltages ranging from 400 to 800 VAC. Numerous other devices require a wide variety of voltages. This conversion process is central to the functioning of an Electric Vehicle. WBGs can perform this task far more efficiently than traditional silicon (Si) semiconductors.
So, How Do Wide Bandgap Semiconductors Work?
Consider accessing a LiB’s voltage through a switch. Turning the switch on and off generates pulsating DC. Electrical engineers know how to convert pulsating DC into a broad spectrum of AC or DC voltages. The efficiency of the switching and the device’s heat handling capability are key.
Let’s compare GaN and SiC with traditional Silicon (Si) semiconductors.
Faster Switching: When converting DC to another DC voltage level, the WGB is instructed to switch ON and OFF thousands of times per second, producing pulsating DC. The process of converting these “pulses” into clean, stable DC requires a “filter” primarily composed of inductors and capacitors. The higher the pulse frequency, the smaller the filter components can be, saving considerable weight and space. Similar benefits are realized when converting DC to the AC required by the main “tractor motor” that replaces a conventional vehicle’s gasoline engine.
WGB semiconductors can switch much faster than silicon devices.
Lower “ON” Resistance: Power semiconductors are often likened to switches, and an ideal semiconductor, like an ideal electromechanical switch, should have as close to zero resistance as possible. Any resistance results in heat generation and power wastage due to voltage crossing resistance. The resistance across the semiconductor, the resistance between the device’s “source” and “drain”, is known as the RSD(ON).
Here, too, WGBs outperform Si devices.
Heat: While WGB semiconductors operate more efficiently than Si devices, some inefficiency inevitably leads to heat generation. However, WBGs can withstand more internal heat and still function safely and efficiently. Moreover, WGBs have greater thermal conductivity, facilitating easier heat dissipation away from the semiconductor.
What are the Main Differences between GaN and SiC?
Two differences are particularly relevant to EV designers. Generally, SiC can handle more power, but GaN can switch faster. The landscape is continually evolving, but this traditional diagram provides a snapshot.
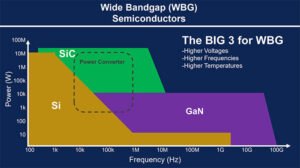
However, there is one aspect where WGBs fall short, and that’s in terms of cost, particularly for SiC.
A key component of an EV is the drivetrain inverter, a semiconductor device that transforms the LiB’s DC voltage into fluctuating AC waveforms needed to drive the EV’s main motor. For more robust EVs, high-power SiC semiconductors are the obvious technical selection. However, as we will discover, the steep price of SiC devices poses a significant hurdle.
Challenges with Tesla’s SiC
As highlighted by PSG Consultancy, Tesla pioneered the adoption of SiC technology for a drivetrain inverter, becoming the first EV manufacturer to do so. This innovation, targeted at the widely popular Model 3, sparked a significant expansion in the SiC industry. It’s worth noting that this had repercussions extending beyond the realm of transportation.
However, in 2023, Tesla made a startling announcement of a planned 75% reduction in SiC usage. Speculations arose that they might switch to more affordable GaN devices or possibly revert to the previous generation Silicon IGBTs.
A closer look at the presumed internals of the Tesla Inverter can provide some clarity.
The fundamental diagram for a DC-to-AC converter reveals that each leg of the Model 3’s inverter uses eight 650V SiC MOSFETs from STMicroelectronics, arranged in parallel. This amounts to a total of 48 semiconductors. While costly, the enhanced efficiency compared to earlier drivetrain inverters allowed the Model 3 to achieve greater range without increasing the LiB’s capacity, size, or weight.
However, the announcement may not be as drastic as it initially appears.
- Optimizing the inverter’s placement within the chassis will enable SiCs to dissipate more heat, allowing each semiconductor to be driven harder and reducing the need for eight on each leg.
- Significant investments are being poured into SiC development, and the emergence of more efficient semiconductors will reduce the number required.
- The anticipated shift to 800V systems will result in lower current and heat due to the higher voltage.
- Future models might feature less powerful motors, thereby requiring fewer SiC semiconductors proportionally.
Nevertheless, the swift progress of more affordable GaN technology could potentially replace SiC in certain design scenarios.
Power Semiconductors made of Gallium Nitride for EV Systems operating at both 400V and 800V.
As highlighted in an earlier Electropages article, GaN-on-silicon takes advantage of existing infrastructure and is usually restricted to 650V. However, the introduction of GaN-on-Qromis-substrate-technology (QST) permits the creation of thicker epitaxial layers, enabling operation at potentially higher voltages, possibly exceeding 1,200V.
This positions GaN semiconductors to power nearly all current EVs and those likely to emerge in the near future. Notably, SiC devices are significantly more challenging to produce, which is reflected in their elevated costs.
Crucially, the switching speeds of GaN are high, even when compared to SiC, and certainly to traditional silicon. This suggests superior efficiency, as well as reduced weight and space requirements.
For these and other reasons, GaN is poised to challenge SiC, particularly in the context of more affordable, mass-market EVs.
Obstacles and Prospects
The primary obstacle to widespread EV adoption is the insufficient charging infrastructure. Recognizing the inevitable shift, gas retailers are logically transitioning to offer profitable charging services for electric vehicles.
Currently, the efficiency of the energy transfer from the charging station to the EV’s wheels exceeds 90% by most estimates. The final significant challenge lies in the LiB itself. Researchers and engineers across the US, Europe, and East Asia are diligently working to address these issues, with the charging time of the device being a primary concern.
While the initial push for WGB development may have been driven by EVs, other technological sectors are not only benefiting from this advancement but also contributing to its progress. These sectors include:
- Internet server farms that require a stable power supply and are experiencing exponential growth due to server-based artificial intelligence.
- Solar and wind energy.
- The smart grid.
- Medical imaging technologies such as MRI machines and CT scanners.
- Radar systems.
- Missile technology.
Conclusion
Wide bandgap semiconductors switch faster, have lower “ON” resistances, and handle heat far better than last-generation silicon devices. They can be found across the whole gamut of electrical and electronic devices – everything from tiny medical wearables to the largest electric vehicles.
Two of the main differences between SiC and GaN semiconductors are that SiC can handle more power, while GaN switches faster and is cheaper. And of critical importance, GaN is easier to manufacture and is consequently less expensive to OEMs.
The previous limitations on the power that GaN devices can handle and in the voltages at which they operate are being gradually overcome, allowing them to challenge SiC’s previous domination in high power EV applications.
References:
- Examining Tesla’s 75% SiC Reduction: https://www.pgcconsultancy.com/post/examining-tesla-s-75-sic-reduction